Authors: Malte Albrecht, Joshua Kossenjans, Mareike Schaumburg
Last updated: October 1st 2023
1. Definition and relevance
Non-renewable fuels and energy refer to energy resources derived from finite reserves that have been formed over geological timescales. These resources, crafted by the Earth’s natural processes, include fossil fuels such as coal, oil, and natural gas, as well as nuclear energy sources like uranium.1 As a result, the stock of non-renewable resources is inherently depletable, setting a stark contrast to their renewable counterparts, such as solar, wind, and hydropower.
A cursory examination of the global energy landscape underscores the dominant role played by non-renewable energy sources. In 2022, the world’s primary energy consumption was around 167,787 terawatt per hour (TWh), of which over 85% came from non-renewable sources. Oil made up the largest part with over 31%, followed by coal with 26% and gas with a share of over 23%. Nuclear energy, on the other hand, accounted for only 4% of global consumption.2
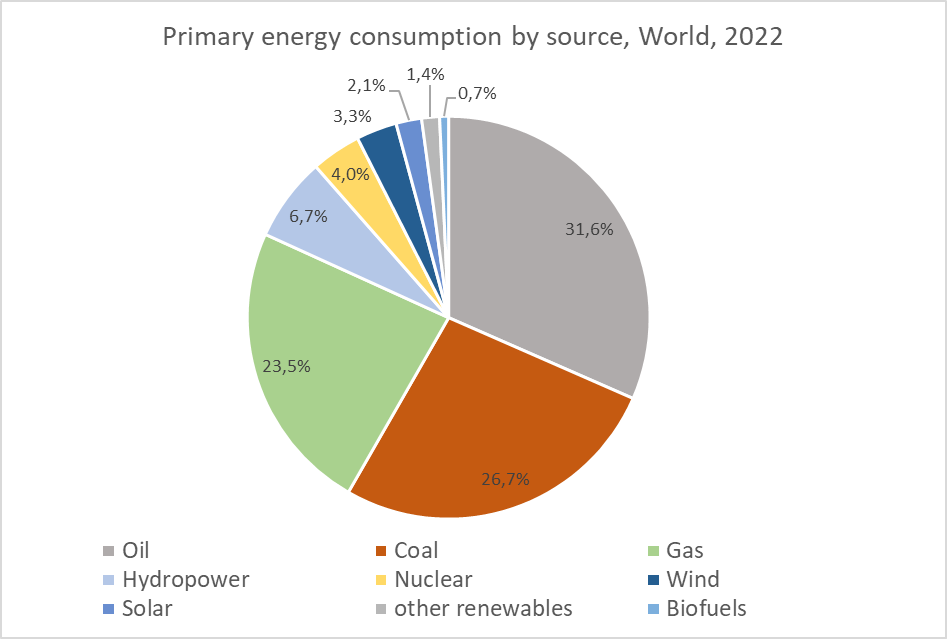
Figure 1: Primary energy consumption by source, World, 2022 (own illustration based on 2)
This energy disparity is further accentuated when dissecting energy consumption across various end-use sectors. While the transport and industrial sectors lean heavily on oil as their primary energy source, other sectors exhibit greater diversity, with a melange of oil, natural gas, electricity, biomass, and coal. The latter remains particularly prominent in heavy industry applications.3
The energy landscape’s future trajectory carries intriguing implications. Despite growing concerns about climate change and a surge in renewable energy adoption, coal is projected to maintain a significant presence, providing 30% of the world’s electricity even in the year 2040.4 This serves as a stark reminder that transitioning to 100% renewable energy within the foreseeable future remains a formidable challenge, necessitating unforeseen technological breakthroughs and due to the economic constraints of transitioning away from certain fuels in energy-intensive industries, which are also referred to as hard-to-abate sectors, like iron and steel, cement, and petrochemicals.5 Natural gas and coal are projected to maintain a substantial role in these sectors, with carbon capture and utilization, which will be expound in Chapter 3, playing a pivotal role in emissions abatement.3
A compelling narrative emerges when considering the interplay between developed and developing economies. In the developed world, coal consumption shows signs of waning, particularly in regions like Europe and North America. However, this decline is offset by a heavy use of coal in developing economies, particularly across Asia, where industrialization and energy demand are on the ascent.5
In advanced economies, nuclear power emerges as a significant low-carbon energy source, contributing 40% of all low-carbon generation and therefore nuclear power provides almost more energy than the doubled amount of solar and wind combined.6 This energy form plays a pivotal role in specific nations, providing more than half of their power requirements. The European Union, for instance, draws 25% of its electricity supply from nuclear reactors, highlighting the technology’s enduring significance in the energy mix.7
This introduction sets the stage for a deeper exploration of non-renewable fuels and energy, a complex and multifaceted domain at the heart of global energy dynamics. It underscores the imperative of addressing the challenges posed by finite resources, the resilience of non-renewable energy sources, and the intricate interplay between developed and developing economies in shaping the future of our energy landscape.
2. Sustainability impact and measures
The relevance of non-renewable fuels and energy to sustainability is a topic of great concern. Sustainability aims to meet the needs of the present generation without compromising the ability of future generations to meet their own needs.8 Non-renewable fuels are inherently unsustainable because they are limited in quantity and will eventually become depleted. Their extraction and use therefore have significant environmental, social, and economic impacts.
2.1. Environmental impact
In 2019, 34% of the world’s greenhouse gas emissions, amounting to 20 gigatons of carbon dioxide (CO2) equivalent, were attributed to the energy sector, making it a pivotal contributor to global climate challenges.9 CO2-equivalent serves as a metric measure that allows us to effectively compare emissions from various greenhouse gases based on their global-warming potential. This comparison is achieved by converting the emissions of different gases into the equivalent amount of carbon dioxide, which possesses the same global warming potential.10 The reason behind the prominence of CO2-equivalent measurements lies in the fact that CO2 and other greenhouse gas (GHG) emissions stand as some of the most pressing challenges in this sector.11 As the world grapples with the consequences of climate change and strives for sustainability, these two indicators, CO2 and GHG emissions, are emerging as suitable measurement tools. They enable us to gauge the environmental impact of various activities, industries, and regions, facilitating informed decisions and actions toward a more sustainable future.
Electricity generation shoulders a substantial portion of the sustainability burden, constituting close to 40% of energy-associated CO2 emissions.4 Its unrelenting reliance on non-renewable fuel sources carries profound implications for the global carbon budget. The prognosis is concerning, as global electricity demand is projected to surge by 60% from 2016 to 2040, driven by the clamour for energy in residential, commercial, industrial, and transportation sectors.4 A large part of the emissions can be traced back to the various sub-sectors. Of the total 34% of emissions that occur in the energy sector, 23% can be indirectly attributed to industry with a large share of 43%, transport with 1.6% and houses with 46.9%. Just 8.5% of this can be assigned to other energy systems.9
If the CO2 emissions are considered after non-renewable energies, 14.98 billion tons of CO2 were emitted by coal in 2021. Oil and gas are responsible for total 19.76 billion tons.12 Coal accounts for over 40% of the growth in overall global CO2 emissions in the same year.13
Population growth emerges as a key catalyst behind the rising energy demand. As the global population swells, the sector grapples with the challenge of meeting this surging energy need while simultaneously curbing its carbon footprint. Global CO2 emissions are anticipated to crest around 10% above 2016 levels by 2040, heralding a pivotal juncture in our quest for sustainability.4
2.2. Social impact
The non-renewable fuels and energy sector harbours multifaceted health and social implications, casting a shadow over the industry’s contributions to global energy needs. A closer examination reveals a spectrum of challenges, from occupational hazards to broader health and societal concerns.
Coal mining, despite a declining rate of fatalities and injuries, remains notorious for its occupational health effects.14 Studies have exposed that up to 12% of coal miners are afflicted with potentially fatal diseases like pneumoconiosis, progressive massive fibrosis, emphysema, chronic bronchitis, and accelerated lung function loss.15 In stark contrast, the health effects stemming from gas have emerged as significantly milder, stemming from both the superior technology employed in Europe and the inherently reduced emissions per unit of energy generated. Oil, while incurring higher health burdens compared to gas, still stands far removed from the toll extracted by coal or lignite. Yet, accidents tied to oil remain elevated, albeit markedly lower than those associated with coal and lignite.14
The realm of nuclear energy introduces a distinct tapestry of health considerations. While occupational deaths are rare in the context of normal operations, risks arise from the intricate nuclear fuel cycle, encompassing mining, routine radiation, decommissioning, reprocessing, and waste disposal. The potential consequences of accidents and non-routine radiation remain contentious, revealing a disparity between expert assessments from the industry and public perceptions. The safety trajectory, though improving since 1990, is weighed against persistent concerns. Unearthing the impacts of uranium exposure on miners uncovers an increased risk of genetic damage, and an associated susceptibility to malignant transformation.14
In the entire supply chain for energy sources, from extraction to processing in power plants, approximately 51.79 people die from accidents and the resulting air pollution for every TWh produced. Coal is the biggest driver with 24.6 deaths per TWh, followed by oil and gas with a total of 21.2. However, it must be remembered that coal accounts for the largest share in the global energy mix. In comparison, the number of deaths from renewable energies such as hydropower, wind and solar is just 1.36. Only nuclear power fares better with 0.03 deaths per TWh. To better illustrate the numbers, one TWh is enough to power a city of 150,000 people. That would mean in one year almost 25 people would die from accidents and the consequences of air pollution, just from coal as an energy resource. For comparison, in solar production, there would be one fatality every 50 years. However, nuclear energy has also emerged as one of the safest sources of energy. Just about every 33 years, one person would die in production, including those killed in the Chernobyl and Fukushima disasters.16
Approximately 59% of middle- and low-income countries still lack access to reliable energy supplies to sustain basic healthcare infrastructure. Fossil energies are on the rise there, and they are relied on.17
The shift from non-commercial to commercial fuels for electricity generation echoes a positive health narrative. Evidenced by developed countries’ experiences across the past century and ongoing transformations in developing nations, this transition yields tangible health benefits, offering respite from the ramifications of non-renewable sources.14
2.3. Economic impact
Energy, often referred to as the lifeblood of an economy, exerts a profound influence on economic development.18 19 The type and scale of energy consumption have far-reaching and intertemporal effects on the growth trajectory of nations.20 This complex relationship between energy and economics underscores the pivotal role played by non-renewable energy sources in shaping global prosperity.
Energy consumption serves as a crucial indicator of economic development, acting as a barometer for a nation’s growth and well-being. From factories churning out goods to the transportation networks that connect societies, nearly all economic activities hinge on a steady supply of energy. It is, in essence, the oxygen that fuels the engines of both developed and developing economies, driving progress, and elevating living standards.20 18
Countries endowed with abundant fossil fuel resources have experienced an economic advantage. Not only have these nations grown at an accelerated pace compared to their non-endowed counterparts, but they have also formed what can be described as a ‘convergence club.’ In this club, lower-income members have managed to grow substantially faster than developed economies, effectively narrowing the economic gap.20 This underscores the pivotal role that fossil fuel resources have played in the economic development of nations over recent decades.
However, this boon comes with a considerable downside. As the world grapples with the imperative to curb fossil fuel use in the face of climate change, resistance emerges from developing countries seeking to harness their valuable fossil fuel resources as part of a long-term growth strategy.20 The allure of these resources is compelling, and the economic benefits they offer are difficult to ignore. Consequently, countries with fossil fuel reserves often prioritize their exploitation and tend to allocate fewer resources to the development of renewable energy sources. The overconsumption of coal and gas energy resources can hinder development, as it exacerbates environmental challenges and contributes to climate change.18 Thus, nations must navigate the fine line between leveraging non-renewable energy sources for economic growth and addressing the pressing need for sustainability and emission reduction.
In the realm of transportation and industry, addressing hard-to-abate sectors becomes imperative. Despite efforts to transition to cleaner energy sources, natural gas and coal are expected to retain a significant role in certain industrial processes in 2050. This is due to the challenges and costs associated with shifting to alternative fuels in hard-to-abate sectors like iron and steel, cement, and petrochemicals. Here, the role of biofuels, low-carbon hydrogen, and hydrogen-derived fuels like methanol and ammonia becomes pivotal, particularly in heavy road transport, aviation, and marine sectors. These alternatives offer pathways to reduce emissions while sustaining industrial processes that require high temperatures.3
3. Practical implementations
3.1. Carbon Capture and Storage (CCS)
Improving the sustainability performance of the non-renewable fuels and energy sector involves adopting various technologies, processes, measures, and tools.
As already mentioned in Chapter 2, exhaust gases are one of the biggest problems in the sector. The CCS approach holds the promise of allowing societies to preserve their existing carbon-based infrastructure while concurrently minimizing the deleterious effects of CO2 on Earth’s climate system.21 At its core, CCS is a methodology devised to apprehend CO2 at the point of generation, subsequently compressing it into a fluid, and storing it. The process encompasses three distinct phases: CO2 capture, CO2 transportation, and CO2 storage.21
In the initial stage, CO2 is captured from fixed point sources, often found within power plants like coal-fired power plants. A panoply of techniques is employed for this purpose, including absorption, adsorption, separation by membranes, and cryogenic separation. Subsequently, the captured gas mixture undergoes compression, transitioning into a liquid and supercritical fluid, rendering it amenable to transportation via pipelines or vessels to storage locations. These storage options encompass geological storage, ocean storage, and mineralization, offering a spectrum of approaches to safeguard this captured carbon.22 CCS is also a technology that supports so called High-Efficiency, Low-Emissions (HELE) coal-fired plants. It refers to advanced technologies used in coal-fired power plants to make them more efficient and environmentally friendly by reducing emissions of pollutants and greenhouse gases. HELE technologies aim to generate more electricity from coal while releasing fewer harmful substances into the atmosphere.23
The implications of CCS are profound. Not only can it usher in a reduction in CO2 emissions stemming from fossil-based energy sources by more than 65%, but it can also serve as a vital hedge for sustaining fossil fuel energy.24 Remarkably, it is postulated that CCS has the potential to contribute significantly, accounting for approximately 16.67% of the total reductions in carbon dioxide emissions required to meet climate goals by 2050.22
Research endeavours have corroborated the environmental benefits of CCS implementation. Observations reveal substantial decreases in atmospheric CO2 emissions, with significant differentials noted when compared to plants where CCS is not employed. Such findings are consistent with the broader consensus that CCS offers environmental and economic indicators.21
However, amid the optimism surrounding CCS, scepticism emerges. Critics assert that CCS techniques merely defer the release of CO2 into the atmosphere, as the gas cannot be stored indefinitely.21 Additionally, concerns arise over the economic viability of processes such as absorption, adsorption, and membrane separation, particularly in situations devoid of regulations or subsidies. Detractors contend that the deployment of CCS technologies could escalate electricity unit prices. Furthermore, studies have highlighted the intensive energy characteristics of CCS technologies, potentially impacting the energy efficiency and operational flexibility of plants where they are employed.14
3.2. Carbon Capture and Utilization (CCU)
Carbon Capture and Utilization represents an innovative approach to tackle the pressing environmental challenge of CO2 emissions by converting them into valuable resources.25 Within the realm of CCU, CO2 is harnessed and employed, either directly or through transformation, in a range of applications, thus diverting it from its traditional role as a greenhouse gas.26 This transformative concept has garnered substantial attention, promoting new avenues for sustainable practices and industries.
One of the distinctive attributes of CCU is its capacity to sequester CO2, offering the prospect of permanent carbon storage.27 26 For example, CO2 can be incorporated into concrete during the curing process, thus mitigating its environmental impact. Similarly, plastics can be enriched with CO2 content, and CO2 can serve as a raw material in cultivating algae. The harvested algae can subsequently be transformed into biofuels, substituting non-biological carbon sources, and fostering sustainability in the energy sector.28
Presently, CO2 finds its primary utilization in the fertilizer industry and the realm of enhanced oil recovery (EOR). However, a paradigm shift is underway, with emerging applications such as the production of synthetic fuels, chemicals, and building aggregates gaining momentum. This shift reflects the evolving perception of CO2 as a potential value-added resource.26 The oil industry has embraced CCU through EOR from mature oil fields, where CO2 plays a pivotal role. EOR, also known as tertiary oil recovery, is a set of advanced techniques and methods employed in the oil industry to extract additional oil from reservoirs. EOR techniques are utilized to extract the remaining oil by altering the properties of the reservoir or the oil itself. There are several methods, including thermal, chemical, microbial and, one of the most widely used methods, gas EOR. Gases, such as CO2, natural gas, or nitrogen, are injected into the reservoir to improve oil recovery. Injected gases can reduce the oil’s viscosity, increase reservoir pressure, and displace oil towards production wells.29 This commercial utilization of CO2 not only enhances CCU deployment but also generates revenue for CCU projects, effectively transforming captured CO2 into an economic resource.28
Approximately 230 million metric tons (Mt) of CO2 are annually employed in various applications. Notably, the fertilizer industry consumes roughly 130 Mt of CO2 for urea manufacturing, while EOR accounts for approximately 80 Mt. However, CCU’s promising avenues predict a shift in these numbers. Projections indicate that around 10 Mt of CO2 per year could be captured for new applications by 2030, with a substantial portion, approximately 7 Mt, being utilized in synthetic fuel production. To align with the goals of a Net Zero Emissions Scenario by 2050, it is imperative that the source of CO2 for utilization shifts toward air or biogenic sources. Currently, this criterion is met for only a portion of the planned CCU capacity for 2030, around 4 Mt CO2 per year in the case of fuels.26
However, it’s crucial to recognize that not all CO2 utilization leads to emissions reduction. The environmental impact hinges on multiple factors, including the CO2 source, the displaced product or service, the carbon intensity of the conversion process, the retention period of CO2 in the product, and the market’s scale for the specific utilization.25
Although the potential climate benefits of CCU are noteworthy, the limited market size for such applications suggests that dedicated storage remains the primary focus for carbon capture, utilization, and storage initiatives.26 Nevertheless, research, development, and demonstration efforts are vital for promoting scalable and competitive CO2-derived products and services over time.
Pioneering projects like the Abu Dhabi CCS plant, commissioned in 2016, herald a future where CCU plays an integral role in transforming emissions into valuable resources. While opportunities exist for further advancements, particularly in coal, CCS and CCU must be extended to various industries, including gas, steel, cement, and chemicals, to expedite its deployment and contribute to a sustainable, low-carbon future.26
3.3. Combined Heat and Power (CHP)
Combined Heat and Power, also known as cogeneration, is a method of energy generation that simultaneously produces electricity and useful heat from a single fuel source. The primary principle behind CHP is to maximize the utilization of the energy content in the fuel, thereby reducing waste and enhancing overall energy efficiency.30
CHP systems typically use a variety of fuel sources, including natural gas, biomass, diesel, or waste heat from industrial processes. This fuel is combusted in a power generation unit, which can be a gas turbine, steam turbine, or reciprocating engine, to produce electricity. In addition to electricity generation, CHP systems capture and utilize the heat generated during the combustion process. This heat, which would otherwise be wasted in conventional power generation, is recovered, and used for various heating applications, such as space heating, hot water production, or industrial processes.31
One of CHP’s benefits lies in its ability to significantly curtail greenhouse gas emissions, a pivotal endeavour in the fight against global warming. Notably, within the European Union, CHP has been a catalyst for change, contributing to a remarkable 15% of the greenhouse gas emission reductions witnessed between 1990 and 2005. This underscores its vital role in mitigating climate change and aligning with international climate goals.31
To comprehend the magnitude of CHP’s potential, consider the vast amount of heat energy potentially released into the environment by conventional power plants. Figures from the International Energy Agency reveal that in 2014, over 77% of the world’s electrical power originated from power plants predominantly reliant on fossil fuels or nuclear heat.32 Yet, their average efficiency of 33% implies that 36,820 TWh of heat energy could have been harnessed for CHP applications.31 This highlights the considerable untapped energy reservoir CHP systems can exploit.
Economic advantages are not left behind in this narrative. CHP presents an opportunity to reduce investments in the power sector by optimizing existing heat and power generation capacity. By providing localized heat and power, CHP systems reduce the need for extensive transmission and distribution system expansion while mitigating the demand for central power stations.31 The IEA’s estimations suggest that promoting CHP could pare down power sector investment requirements by an impressive 7% between 2005 and 2030.32
As the world inches closer to the 22nd century, there remains a considerable reservoir of untapped heat energy that CHP systems can harness, albeit within technical and temporal limits. Yet, it’s vital to recognize that CHP’s contribution to reducing CO2 emissions hinges on various factors, including the share of renewable energy in the electricity and heat market and the remaining emissions budget. As the proportion of renewables in the electricity and heat market increases and the remaining emissions budget diminishes, the potential for CHP to mitigate CO2 emissions gradually decreases.33 To sustain its role in the energy landscape, CHP must evolve. This holds true for CHP plants utilizing fossil fuels as well as those powered by renewable resources. While CHP plants are poised to replace non-CHP fossil-fuel power plants and play a pivotal role in emissions reduction by 2030, they must pivot towards renewable fuels after this period.30 The march toward renewable energy dominance, coupled with finite renewable fuel resources, calls for a careful balancing act.
Despite its benefits, global CHP adoption has not witnessed significant advancement, with estimates suggesting stabilization at approximately 9% of total generating capacity by the end of the first decade of the 21st century. This underscores the imperative of amplifying efforts to unlock CHP’s full potential in reshaping our energy landscape towards a more sustainable use of non-renewable energy and fuels.30
3.4. Tools and practices
3.4.1. AI technologies: Example Saudi Aramco
Utilizing advanced AI technologies and reservoir modelling simulations, Saudi Aramco, one of today’s biggest players in the oil and energy industry, is effectively enhancing efficiency and risk management within the oil sector. The company is actively developing comprehensive reservoir models that provide insightful guidance into underground structures and their dynamics. By generating diverse data sources and harnessing AI-driven simulations, Saudi Aramco aims to optimize resource extraction, mitigate operational risks, and facilitate well-informed decision-making. This initiative not only increases sustainability in operational contexts but also helps with the strategic planning concerning future excavation areas. Moreover, the application of such simulations extends to potential uses like carbon capture and storage within abandoned mines. The simulations enable Saudi Aramco to predict impacts of storage underground and therefore limiting potential risks and providing a more precise estimation of long-term impacts. This innovative approach harnesses cutting-edge technology, driving sustainable progress throughout the non-renewable energy landscape. Importantly, the versatility of modelling and simulations has the potential to extend across various excavation, storage, and utilization scenarios within the discussed non-renewable sectors.34 Moreover, this approach could yield numerous of positive spillover effects and can also help promoting other innovative ideas, that can be estimated and engineered more precisely with the help of AI and simulations.
3.4.2. Rating system: Example India
Another illustration of initiatives boosting sustainability in the non-renewable fuel and energy sector is shown by the Indian Ministry of Coal. The ministry has showcased the effectiveness of mandatory dust suppression systems, such as fog cannons, during excavation processes, coupled with pre-planned strategies for the rehabilitation of excavation areas, to substantially enhance the coal mining industry’s sustainability. Furthermore, an innovative approach involves the introduction of a rating system for mines in India. These mines are evaluated across multiple dimensions that reach further than just conventional aspects like economic performance and safety measures. The score criteria also include the planned rehabilitation processes, worker compliance, and environmental considerations, thereby incentivizing not just economic best practices, but also practices that strengthen the industry’s social and environmental performance. The ministry aims to acknowledge and spotlight the exemplary performers. Consequently, utilizing top-graded entities as role models for others, hoping to enhance the whole industries footprint on society, the environment, and the markets alike.35
3.4.3. Utilizing geothermal energy
Repurposing the geothermally heated water found in abandoned coal mines for heating or power generation represents an innovative solution that holds substantial advantages for both society and the environment, particularly in regions that suffered from the negative external effects of mining and excavation. Beyond economic benefits, repurposing abandoned mines for geothermal energy aligns with environmental restoration. A fundamental advantage lies in the reduction of carbon emissions. Geothermal energy stands as a low-carbon power source, emitting significantly fewer greenhouse gases than fossil fuels. By utilizing the geothermal heat embedded within abandoned mines, communities contribute directly to local and global initiatives aimed at mitigating climate change. Moreover, this transition increases energy security by diversifying the energy mix, making communities less dependent on external energy sources, and enhancing their resilience against energy market disturbances. By reclaiming underutilized land and transforming it into productive assets, this approach not only promotes sustainable development but also helps regions that once were defined by coal-related activities. Some small-scale case studies like Matas-Escamilla et al. (2023) have shown the potential benefits that this innovation might bring to the coal and mining industry. However, this method might not be applicable for every excavation area due to the fact that the potential energy yield would not be outweighing the upfront and maintenance costs. Therefore, further research into this topic and precise planning will be required for this innovation to increase the mining industries sustainability. Finally, this approach could be potentially aided by other innovative ideas like the before mentioned use of AI and simulations.36
4. Drivers and barriers
4.1. Economic drivers
In the realm of non-renewable energy sources, economic factors play a crucial role in shaping the path towards greater sustainability. The economic drivers differ across these sectors, but a common thread is the need to balance energy and fuel demands with environmental responsibilities. While these non-renewables have inherent challenges due to their finite nature and environmental impacts, they can still strive for sustainability through various approaches.
4.1.1. Oil sector
The oil industry faces challenges stemming from price volatility37, geopolitical pressures, and concerns over emissions.38 Achieving sustainability involves focusing on cleaner extraction techniques, refining processes, and promoting the adoption of alternative fuels. By investing in research and innovation, the industry can transition towards producing lower-carbon fuels and integrating advanced technologies, such as CCS, to offset emissions. The usage of artificial intelligence and geological science can offer a substantial enhancement to the sustainability in the oil and gas sector. Through the generation of models and simulations depicting excavation areas, risks to groundwater can be minimized. This approach not only enhances mining efficiency but also provides a comprehensive understanding of the future utilization possibilities for these areas. For instance, they could be transformed into potential carbon capture and storage facilities, showcasing the multifaceted benefits of integrating advanced technologies.34
4.1.2. Gas sector
Natural gas, often considered a cleaner alternative than most other non-renewables, can enhance its sustainability by addressing methane emissions throughout the supply chain. Especially monitoring and data gathering seem to be promising approaches to enhance current process to become more sustainable and efficient. Technological advancements can help minimize leaks during extraction, transportation, and distribution, for example new monitoring approaches that detect fugitive leaks much quicker and more reliably.39 The industry can also invest in scalable CCS technologies to further reduce emissions. Economic incentives for these innovations could drive the sector towards a more sustainable path.
4.1.3. Coal sector
Coal faces the challenge of being the most carbon-intensive energy source. This derives from the fact that coal is not only a major factor in electricity generation but also is very important in certain hard-to-abate industries like the metal or cement production.3 Striving for sustainability requires focusing on cleaner and more efficient coal combustion technologies, such as HELE power plants.40 Moreover, investment in coal mine reclamation and environmental restoration, can mitigate the industry’s environmental impact, as seen in the Indian projects. These projects also propose an innovative approach in that the Indian government implemented a scoring system in order to rate economic, social and environmental impact and performance of the partaking mines. This score system promotes mines to implement best practices and acknowledge their responsibilities.35 Other approaches concentrate on limiting the negative lasting impacts of excavation and mining endeavours by considering options reaching further than just restoration and reclamation. The use of geothermally heated mine water shows a new promising innovation in a seemingly unsustainable sector and could help especially reducing the negative socioeconomic impacts of mining operations in their respected regions.41 42 36
4.1.4. Nuclear energy
Nuclear energy offers low-carbon electricity generation but comes with concerns related to radioactive waste disposal and safety. To enhance sustainability, innovation is key. Advancements in nuclear reactor designs, including smaller modular reactors and thorium-based reactors, could address safety and waste issues. Research into advanced fuel cycles and technologies for recycling nuclear waste could contribute to the sector’s long-term sustainability.7
4.1.5. Common pathways
Across these non-renewable energy sectors, the pursuit of sustainability centers on several common pathways:
Efficiency improvements: Enhancing energy efficiency in extraction, production, and utilization processes can reduce waste, resource consumption, and associated emissions.
Innovative technologies: Investing in research and development of pioneering technologies, like carbon capture, utilization, and storage, holds the potential to notably curtail emissions and prolong the sustainability of these energy sources. Additionally, the innovative application of artificial intelligence and geological science can diminish adverse effects associated with excavation and drilling operations.
Diversification and transition: Transitioning towards more sustainable energy sources, whether through a blend of non-renewables and renewables or the gradual adoption of alternative fuels, can balance economic interests with environmental responsibilities.
Environmental stewardship: Investing in reclamation, habitat restoration, and responsible waste management demonstrates a commitment to minimizing the environmental impact of energy production.
In conclusion, economic drivers serve as the driving force propelling the non-renewable energy sectors toward sustainability. Through the adoption of efficiency enhancements, innovative technologies, diversification, and environmental stewardship, these industries can effectively address the obstacles posed by resource constraints and environmental considerations. However, achieving this requires a recognition of the crucial role non-renewables play as significant energy and fuel providers. Dismissing them due to perceived environmental shortcomings compared to renewables overlooks their vital contribution and potential for improvements in sustainability. It is essential for both consumers and producers to acknowledge their significance and potential for positive change within the broader energy landscape.
4.2. Political drivers
Political factors significantly shape the direction of non-renewable energy sectors in terms of sustainability. Balancing energy security, economic growth, and environmental concerns requires strategic policy decisions. While these non-renewables face inherent challenges, political drivers can play a pivotal role in driving them towards more sustainable practices.
4.2.1. Oil sector
Political pressures to reduce carbon emissions and enhance energy security are driving the oil industry towards sustainability. Governments can incentivize research into cleaner extraction methods and support the development of renewable alternatives. Strengthening regulations on emissions and fostering international collaboration can encourage the industry to adopt cleaner practices.
4.2.2. Gas sector
Political commitments to emission reduction and cleaner energy sources create momentum for natural gas to evolve sustainably. Governments can promote stricter methane monitoring and regulation to mitigate emissions. Support for CCS infrastructure and incentives for adopting greener gas extraction and utilization practices can drive the sector’s sustainability.
4.2.3. Coal sector
Political choices concerning air quality and climate change have precipitated the decrease of coal consumption in numerous advanced economies.5 To ensure an equitable transition for communities reliant on coal, governments can play a pivotal role by investing in renewable energy initiatives and offering assistance for workforce retraining. Furthermore, the promotion of innovation and technological advancements across various sectors may compel the coal industry to follow suit in order to maintain competitiveness both economically and environmentally. The implementation of policies that incentivize research into cleaner coal technologies and carbon capture can also bolster sustainability endeavours. Evidenced by the Indian projects, policies possess the potential to not only catalyse enhanced efficiency but also foster a heightened sense of responsibility in industrial operations.35
4.2.4. Nuclear energy
Endorsement from policymakers for low-carbon energy sources places nuclear energy in a favourable position. Governments can expedite regulatory procedures for nuclear projects, all the while guaranteeing stringent safety protocols. However, as outlined in chapter 4.5.4, the nuclear sector contends with substantial financial and organizational challenges that impede research and innovation progress.7 In response, policymakers hold the capacity to foster international collaboration in nuclear research and development, not only addressing the hindrances related to financial constraints but also tackling the complexities of waste disposal and safety apprehensions. This collaborative effort paves the way for a sustainable future in nuclear energy.
4.2.5. Common pathways
Several common pathways can help non-renewable energy sectors align with political drivers for sustainability:
Emission reduction commitments: Incorporating emission reduction targets and other guidelines into policy frameworks can guide industries to transition towards cleaner practices and technologies. For instance, the European Union enforces legal obligations on its member states to adhere to specific sustainability objectives and timelines outlined in the European Green Deal. Additionally, the EU mandates significant industries to conduct regular energy audits within its member nations.4
Research and development support: Governments can allocate funding for research and innovation to develop breakthrough technologies that enhance energy efficiency and reduce environmental impact. Given the persistent significance of these industries, as shown in chapter 1, it is imperative not to underestimate but rather advocate for research and development within the non-renewable sector. Especially global collaborative research should be promoted by policies and incentives in order to strive for more sustainability in these sectors.5 43 39 Demonstrated by innovative initiatives like harnessing geothermal energy from abandoned coal mines, research and development can catalyse the emergence of technologies and concepts that amplify efficiency while also mitigating the enduring adverse impacts associated with these industries.42 41 36
Policy incentives: Offering financial incentives, tax breaks, and subsidies for sustainable practices and cleaner technologies can encourage the adoption of more environmentally friendly processes. Nonetheless, the existence of financial and administrative obstacles in certain sectors acts as a deterrent, constraining progress in terms of sustainability enhancements.
In conclusion, political drivers play a pivotal role in shaping the sustainability trajectory of non-renewable energy sectors. By embracing emission reduction targets, supporting research and innovation, providing policy incentives, and fostering international collaboration, governments can guide these sectors towards more sustainable practices, ultimately contributing to a greener energy landscape.
4.3. Social drivers
Social dynamics play a significant role in shaping the sustainability of non-renewable energy sectors. Public awareness, attitudes, and demands for cleaner energy sources can influence industry practices. While these non-renewables face challenges, aligning with societal values can drive them toward more sustainable practices.
4.3.1. Oil sector
Public concern over climate change and air quality is prompting the oil industry to address its environmental impact. To enhance sustainability, oil companies can invest in research and promotion of low-carbon fuel alternatives44 45, improve transparency about emissions46, and engage with communities to mitigate negative social perceptions.
4.3.2. Gas sector
Natural gas, touted as a cleaner alternative, can benefit from societal preferences for lower-emission energy sources. Industry efforts to minimize methane leaks39 and promote the environmental benefits of natural gas47 can align with public desires for cleaner energy options, bolstering its sustainability image.
4.3.3. Coal sector
Societal awareness of air pollution and carbon emissions is driving coal’s decline especially in developed economies.5 48 The coal industry can work toward sustainability by investing in technologies that reduce emissions, actively participating in community dialogues, and collaborating with stakeholders to ensure responsible mine reclamation and environmental restoration.40 35
4.3.4. Nuclear energy
Public perceptions of nuclear energy as a low-carbon source can drive support for its sustainability. The industry can enhance its social acceptance by prioritizing safety, transparent waste management, and actively engaging with communities.7
4.3.5. Common pathways
Several common pathways can align non-renewable energy sectors with social drivers for sustainability:
Public engagement: Engaging with communities, stakeholders, and the public to understand concerns, share information, and incorporate feedback can foster a more positive social image.49
Transparency: Openly communicating about environmental impacts, safety measures, and emissions reduction efforts can build trust and align with social values.
Benefits to society: Non-renewable energy sources ensure energy security and well-being by providing stable and reliable power for essential services and industries during the transition to sustainable alternatives, contributing to economic stability and improved quality of life.14 5
4.4. Economic barriers
4.4.1. Oil sector
Fluctuating economic conditions37 and infrastructure expenses50 impede the advancement of sustainability in the oil sector. The promise of CCS as a means to bolster sustainability is constrained by the requisite technological shift needed for optimal CCS implementation.21
4.4.2. Gas sector
The gas sector struggles with economic barriers stemming from fluctuating market prices51 and substantial initial investments, particularly in areas like transportation.52 Furthermore, inadequate global research and development initiatives within the sector hinder the potential for technological breakthroughs that could lead to economic, social, and various other advantages.43
4.4.3. Coal sector
Coal, as an energy source, has the advantage of affordability and a straightforward initial technology setup. However, this leads to a significant economic barrier for the sectors sustainability. Particularly in developing nations, the appeal lies in utilizing this cost-effective and accessible resource to meet rising energy demands during periods of economic growth. Overlooking the potential environmental and societal issues of coal mining and usage in the long run leads to coal being the cheapest and easiest to scale energy source for said countries.5
4.4.4. Nuclear energy
Nuclear energy faces economic barriers primarily in the form of financial and organizational challenges. The need for reactor refurbishments is mentioned, yet political, societal and economic risks limit new investment capital required for maintaining efficiency and safety. The sector further struggles with the recent poor economic performance of investments, shown by significant cost overruns, reportedly exceeding 300 percent of initial projections, and delays exceeding five years due to organizational complications. These factors discourage potential investors who would be essential for maintaining and enhancing the sector’s sustainability.7
4.4.5. Common pathways
Across diverse non-renewable energy sectors, common economic barriers emerge as formidable challenges. Volatile market conditions especially for the gas and oil market, high upfront costs of infrastructure, and financial constraints hinder the transition towards sustainable practices. These barriers hinder the adoption of cleaner technologies and slow progress towards reduced environmental impact. In conclusion, the journey towards sustainability for non-renewable energy sectors necessitates overcoming economic barriers through a strategic blend of efficiency improvements, innovative technologies, diversification, and collaborative efforts. By leveraging these pathways, these industries can achieve economic prosperity with environmental responsibility, driving the evolution towards a more sustainable energy landscape.
4.5. Political barriers
Across all mentioned non-renewable energy and fuel sectors, political barriers pose significant challenges. Some countries forbid investment in certain industries since their goal is a quick transition towards renewable energy sources or because public demand and acceptance is greater for these types of energies. This lasting global shift towards renewable energy sources has led to political focus on these alternatives, often disregarding investment in research and development within non-renewable sectors. To bridge this gap, fostering especially global collaborative research and development efforts emerges as a promising solution. Developing nations could benefit from assistance and knowledge-sharing by more developed countries in optimizing their non-renewable energy usage like the more common coal sectors in these countries. By collectively addressing these political barriers and placing renewed emphasis on technological progress and innovation in the sector of non-renewable energy and fuel, a more balanced and sustainable energy landscape could be built. This landscape focusses not only on future use and consumption of renewables but will also acknowledge the fact that non-renewables will play an important role in the transition.5 43 3
4.6. Social barriers
Across all non-renewable energy sectors, shared social barriers show a significant impact. Public perception, often shaped by environmental concerns, has considerable influence over industry practices and policies.49 As the popularity of renewable alternatives increases, non-renewable sectors face challenges in keeping public support for sustainable initiatives and development. This can be seen in the already mentioned non-sufficient investments in industries burdened with negative environmental and societal associations. However, this could be offset by the before mentioned drivers of sustainability. Increased engagement of the industries in social initiatives, a more established way of transparency39 46 and a general acknowledgment of the sectors importance could help to limit these burdens and the reputation issues.
In conclusion, addressing social barriers and achieving sustainability in non-renewable energy sectors involves aligning industry practices with public concerns, enhancing transparency, and actively engaging with communities. By embracing these pathways, these sectors can bridge the gap between societal values and industry practices, driving the transition towards a more sustainable energy landscape.
5. Recommendations
Taking the recommendations into consideration, we intend to augment the existing wiki content related to corporate philanthropy. As the wiki aims to provide a concise overview for academic purposes, we propose the addition of a brief paragraph beneath the definition section:
It is important to acknowledge the expansive nature of the philanthropy sector and its literature. Specifically, the term “strategic philanthropy” can potentially lead to confusion if not further elaborated. A literature review conducted by Haydon et al. (2021) highlights the widespread use of terms like “strategic” within the context of philanthropy, often interchangeably and with varying interpretations. The review observes a phenomenon termed “philanthrocapitalism,” illustrating the lack of coherence and consistency in the labelling of philanthropic practices. 53 Michael Edwards (2008) also addressed this inconsistency as early as 2008. 54 Rogers (2011) underscores how this inconsistency burdens scholarly and scientific discussions within the sector. 55
The intention behind incorporating this paragraph is to assist future students and researchers in navigating the topic of corporate philanthropy without confusion. By providing this information upfront, we aim to guide their deeper literature reviews, armed with the awareness that the literature may encompass diverse descriptions and terminologies.
Furthermore, an additional aspect to consider for this subject is the potential inclusion of information in chapters “3.3 Planning and controlling” and “3.4 Measuring instruments.” This content could highlight the complexity of measuring and assessing the true outcomes of philanthropic activities, a challenge underscored by several scholars including the previously mentioned Edwards (2008).54 Jenkins (2011) also delves into this matter and even provides instances of misinterpretation in measurement, particularly problematic when the goal is to achieve a social benefit.56
Another small recommendation for the existing wiki is regarding the topic of “Carbon Accounting (GHG Protocol)”. According to the GHG Protocol Corporate Standard reporting of Scope 3 emissions are optional and rather seen as a possibility for the reporting organization or company to be innovative in greenhouse gas management. For us, this is a relevant detail of the GHG Protocol as it means that Scope 3 emissions are difficult to compare between different companies. The optionality of Scope 3 emissions also plays a role when setting the operational boundaries. It must be determined what Scope 3 emissions are relevant in terms of value-creation or in terms of ecological impact as to get a best possible overview of the company’s impact.57
References
1. Groth, C. A New-Growth Perspective on Non-Renewable Resources. in Sustainable Resource Use and Economic Dynamics (eds. Bretschger, L. & Smulders, S.) 127–163 (Springer Netherlands, 2007). doi:10.1007/978-1-4020-6293-3_7.
2. Ritchie, H., Roser, M. & Rosado, P. Energy. Our World in Data https://ourworldindata.org/energy-mix (2022).
3. bp. bp Energy Outlook 2023. (2023).
4. Zohuri, B. & McDaniel, P. J. Introduction to energy essentials: insight into nuclear, renewable, and non-renewable energies. (Adademic Press, 2021).
5. The Long Goodbye: Why Some Nations Can’t Kick the Coal Habit. Kleinman Center for Energy Policy https://kleinmanenergy.upenn.edu/research/publications/the-long-goodbye-why-some-nations-cant-kick-the-coal-habit/ (2023).
6. International Energy Agency. Nuclear Power in a Clean Energy System. Int. Energy Agency (2019).
7. International Energy Agency. Nuclear Power in a Clean Energy System – Analysis. IEA https://www.iea.org/reports/nuclear-power-in-a-clean-energy-system (2019).
8. Agnelli, S. Unsere gemeinsame Zukunft: d. Brundtland-Bericht. (Eggenkamp, 1987).
9. Emissions Trends and Drivers. in Climate Change 2022 – Mitigation of Climate Change (ed. Intergovernmental Panel On Climate Change (Ipcc)) 215–294 (Cambridge University Press, 2023). doi:10.1017/9781009157926.004.
10. eurostat. Glossary:Carbon dioxide equivalent. https://ec.europa.eu/eurostat/statistics-explained/index.php?title=Glossary:Carbon_dioxide_equivalent.
11. Friedlingstein, P. et al. Global Carbon Budget 2022. Earth Syst. Sci. Data 14, 4811–4900 (2022).
12. Ritchie, H., Roser, M. & Rosado, P. CO₂ and Greenhouse Gas Emissions. Our World in Data https://ourworldindata.org/emissions-by-fuel (2020).
13. IEA. Global Energy Review: CO2 Emissions in 2021. (2021).
14. Markandya, A. & Wilkinson, P. Electricity generation and health. The Lancet 370, 979–990 (2007).
15. Stephens, C. & Ahern, M. Worker and Community Health Impacts Related to Mining Operations Internationally A Rapid Review of the Literature. (2001).
16. Ritchie, H. What are the safest and cleanest sources of energy? Our World in Data https://ourworldindata.org/safest-sources-of-energy.
17. Romanello, M. et al. The 2022 report of the Lancet Countdown on health and climate change: health at the mercy of fossil fuels. The Lancet 400, 1619–1654 (2022).
18. Wang, H. et al. Fossil Energy Demand and Economic Development in BRICS Countries. Front. Energy Res. 10, (2022).
19. Zou, C., Zhao, Q., Zhang, G. & Xiong, B. Energy revolution: From a fossil energy era to a new energy era. Nat. Gas Ind. B 3, 1–11 (2016).
20. Oliver, M. E. & Upton, G. B. Are Energy Endowed Countries Responsible for Conditional Convergence? Energy J. 43, (2022).
21. Pires, J. C. M. Carbon Capture and Storage. (MDPI – Multidisciplinary Digital Publishing Institute, 2019). doi:10.3390/books978-3-03921-400-6.
22. International Energy Agency. Technology Roadmap: Carbon Capture and Storage. (OECD Publishing, 2013).
23. International Energy Agency. Technology Roadmap – High-Efficiency, Low-Emissions Coal-Fired Power Generation – Analysis. IEA https://www.iea.org/reports/technology-roadmap-high-efficiency-low-emissions-coal-fired-power-generation.
24. Global Energy Assessment (GEA). (Cambridge University Press ; International Institute for Applied Systems Analysis, 2012).
25. Ostovari, H., Sternberg, A. & Bardow, A. Rock ‘n’ use of CO2: carbon footprint of carbon capture and utilization by mineralization. Sustain. Energy Fuels 4, 4482–4496 (2020).
26. International Energy Agency. Carbon Capture, Utilisation and Storage – Energy System. IEA https://www.iea.org/energy-system/carbon-capture-utilisation-and-storage.
27. Gabrielli, P., Gazzani, M. & Mazzotti, M. The Role of Carbon Capture and Utilization, Carbon Capture and Storage, and Biomass to Enable a Net-Zero-CO2 Emissions Chemical Industry. Ind. Eng. Chem. Res. 59, 7033–7045 (2020).
28. World Coal Association. Clean coal. World Coal Association https://www.worldcoal.org/clean-coal-technologies/clean-coal-2/ (2023).
29. Thomas, S. Enhanced Oil Recovery – An Overview. Oil Gas Sci. Technol. – Rev. IFP 63, 9–19 (2008).
30. BMWK. Combined heat and power. https://www.bmwk.de/Redaktion/EN/Artikel/Energy/modern-power-plant-technologies.html.
31. Breeze, P. A. Combined Heat and Power. (Elsevier, 2018). doi:10.1016/C2016-0-03590-8.
32. International Energy Agency. Key World Energy Statistics 2016. (OECD, 2016). doi:10.1787/key_energ_stat-2016-en.
33. Sensfuß, D. F., Deac, G. & Bernath, C. Vorabanalyse Langfristige Rolle und Modernisierung der Kraft-Wärme-Kopplung. (2017).
34. armaco. How can today’s resources fuel our shared tomorrow? https://www.aramco.com/ https://www.aramco.com/en/campaigns/powered-by-how/reservoir-modelling (2023).
35. Ministry of Coal, Government of India. SUSTAINABILITY IN COAL MINES. https://coal.nic.in/en/public-information/reports/annual-reports/annual-report-2022-23 (2023).
36. Matas-Escamilla, A. et al. Mine water as a source of energy: an application in a coalfield in Laciana Valley (León, NW Spain). Clean Technol. Environ. Policy (2023) doi:10.1007/s10098-023-02526-y.
37. Yépez-García, R. A. & Dana, J. Economic Effects of High and Volatile Oil Prices. in Mitigating Vulnerability to High and Volatile Oil Prices 19–27 (The World Bank, 2012). doi:10.1596/9780821395776_CH02.
38. International Energy Agency. Oil 2023 – Analysis. IEA https://www.iea.org/reports/oil-2023 (2023).
39. Erland, B. M., Thorpe, A. K. & Gamon, J. A. Recent Advances Toward Transparent Methane Emissions Monitoring: A Review. Environ. Sci. Technol. 56, 16567–16581 (2022).
40. Ali, H., Phoumin, H., Weller, S. R. & Suryadi, B. Cost–Benefit Analysis of HELE and Subcritical Coal-Fired Electricity Generation Technologies in Southeast Asia. Sustainability 13, 1591 (2021).
41. Kretschmann, J. Post-Mining—a Holistic Approach. Min. Metall. Explor. 37, 1401–1409 (2020).
42. Geospatial Research Ltd. Mine-Water Heat. Geospatial Research https://geospatial-research.com/mine-water-heat/ (2023).
43. Al-Yafei, H. et al. A systematic review for sustainability of global liquified natural gas industry: A 10-year update. Energy Strategy Rev. 38, 100768 (2021).
44. Environmental and Energy Study Institute. Alternative Low / No Carbon Fuels | EESI. https://www.eesi.org/topics/alternative-fuels/description (2023).
45. Wang, Y. et al. A review of low and zero carbon fuel technologies: Achieving ship carbon reduction targets. Sustain. Energy Technol. Assess. 54, 102762 (2022).
46. Jarvis, M. Transparency in the Petroleum Sector: The Importance of Information and Clear Processes for Effective Development in Fragile Contexts. in Balancing Petroleum Policy: Toward Value, Sustainability, and Security 151–168 (The World Bank, 2019). doi:10.1596/978-1-4648-1384-9_ch7.
47. U.S. Energy Information Administration. Natural gas and the environment – U.S. Energy Information Administration (EIA). https://www.eia.gov/energyexplained/natural-gas/natural-gas-and-the-environment.php (2022).
48. International Energy Agency. Coal Market Update_July 2023. Int. Energy Agency (2023).
49. Peterson, T. R., Stephens, J. C. & Wilson, E. J. Public perception of and engagement with emerging low-carbon energy technologies: A literature review. MRS Energy Sustain. 2, E11 (2015).
50. Favennec, J.-P. Economics of Oil Refining. in The Palgrave Handbook of International Energy Economics (eds. Hafner, M. & Luciani, G.) 59–74 (Springer International Publishing, 2022). doi:10.1007/978-3-030-86884-0_3.
51. Directorate-General for Energy. High volatility and geopolitical tensions impact electricity and gas market developments in Q1 2022. https://commission.europa.eu/ https://commission.europa.eu/news/high-volatility-and-geopolitical-tensions-impact-electricity-and-gas-market-developments-q1-2022-2022-07-08_en (2022).
52. Molnar, G. Economics of Gas Transportation by Pipeline and LNG. in The Palgrave Handbook of International Energy Economics (eds. Hafner, M. & Luciani, G.) 23–57 (Springer International Publishing, 2022). doi:10.1007/978-3-030-86884-0_2.
53. Haydon, S., Jung, T. & Russell, S. ‘You’ve Been Framed’: A critical review of academic discourse on philanthrocapitalism. Int. J. Manag. Rev. 23, 353–375 (2021).
54. Edwards, M. Just another emperor? the myths and realities of philanthrocapitalism. (Demos, 2008).
55. Rogers, R. Why Philanthro-Policymaking Matters. Society 48, 376–381 (2011).
56. Jenkins, G. Who’s Afraid of Philanthrocapitalism ? Case West. Reserve Law Rev. 61, 753 (2011).
57. The greenhouse gas protocol: a corporate accounting and reporting standard. (World Business Council for Sustainable Development ; World Resources Institute, 2004).